First experimental demonstration of a new type of space-based gravitational wave detector: Toward the realization of high-sensitivity observation in the 0.1 Hz band
Feb. 26, 2025 | GATEWAY to Academic Articles
The detection of gravitational waves, which are ripples in space-time, allows us to obtain a variety of astronomical and physical insights. Like electromagnetic waves, gravitational waves are also expected to allow us to observe various different phenomena in each frequency band. In particular, observing gravitational waves in frequency bands lower than around 10 Hz, which are challenging to detect with current ground-based detectors, is expected to yield a wealth of novel scientific insights. In order to achieve high-sensitivity observations of such low-frequency gravitational waves, a space-based gravitational wave detector is under development. At present, the most common method for detecting gravitational waves is to use a laser to measure variations in the distance between mirrors. In particular, detectors that use optical cavities can achieve high sensitivity even with relatively low laser power. A Fabry–Pérot cavity (hereafter called a cavity) is a mechanism with two mirrors facing each other, and by controlling the distance between the mirrors and the laser frequency, a resonance phenomenon occurs, enhancing the light inside the cavity. The Fabry–Pérot cavity-type detector utilizes the fact that the mirrors of the cavity in resonance have a high sensitivity to displacement. The Fabry–Pérot cavity-type detectors have to maintain the resonance during observation. Using this conventional method for a space-based detector, spacecraft fly in synchronized formation carrying the mirrors with the cavity being the distance between the spacecraft. The length between the spacecraft have to be controlled with ultra-high precision. While efforts continue to achieve such precise control, a method called the back-linked Fabry–Pérot interferometer (BLFPI) has been proposed as an alternative approach in recent years. BLFPI allows for maintaining cavity resonance using only the laser frequency. As a result, in principle, ultra-precise control of the cavity length can be avoided. On the other hand, BLFPI faces the challenge of sensitivity degradation due to the laser frequency noise. To address this issue, it was proposed to “subtract” frequency noise from the acquired signals through post-processing. However, this had not yet been experimentally demonstrated. In this study, we constructed a BLFPI setup on a tabletop and demonstrated the subtraction of the frequency noise. In this demonstration, we achieved a maximum noise reduction ratio of approximately 200. Furthermore, by analyzing the factors limiting the noise reduction ratio, we outlined a path for further noise reduction in the future. This is the first experimental demonstration of the frequency noise subtraction technique, which is essential for achieving high sensitivity in the BLFPI.
Research Summary
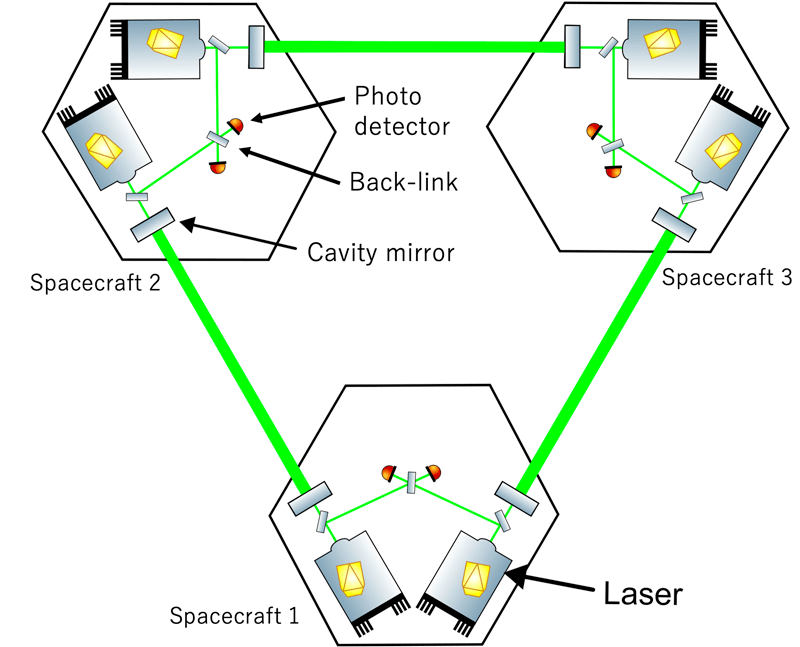
By realizing highly sensitive observations of gravitational waves in the 0.1-10 Hz band, we can expect to obtain a wealth of scientific results*1. To achieve such observations, a space-based detector*2 utilizing the Fabry–Pérot cavities*3 has been proposed. A Fabry–Pérot cavity is an interferometric device that resonates light between the mirrors. We colloquially call the Fabry–Pérot interferometer the Fabry–Pérot cavity or cavity in short, hereafter. Resonance occurs when the distance between the mirrors is an integer multiple of half the wavelength of the incident laser. In the resonant state, the cavity exhibits high sensitivity to fluctuations in the laser frequency or the displacement of the mirror. Optical cavity detectors leverage this property to detect fluctuations in the cavity length caused by gravitational waves, allowing for high sensitivity with relatively low laser power. A representative project employing optical cavity detectors is Japan’s DECIGO.
Space-based optical cavity detectors are planned to construct three optical cavities between satellites, as shown in Figure 1. The method studied in this research, called the BLFPI (back-linked Fabry–Pérot interferometer) *4, equips each satellite with two lasers. The frequency of each laser is controlled to follow the resonant frequency determined by the cavity length; in other words, the information about the cavity length is copied to the laser frequency. By interfering with the lasers sent in opposite directions and extracting the "beat" signal, the difference of the frequency between the lasers, i.e., the differential length of the two cavities, can be obtained. The gravitational waves stretch one cavity length and compress the other, allowing gravitational wave signals to be extracted from the beat signal.
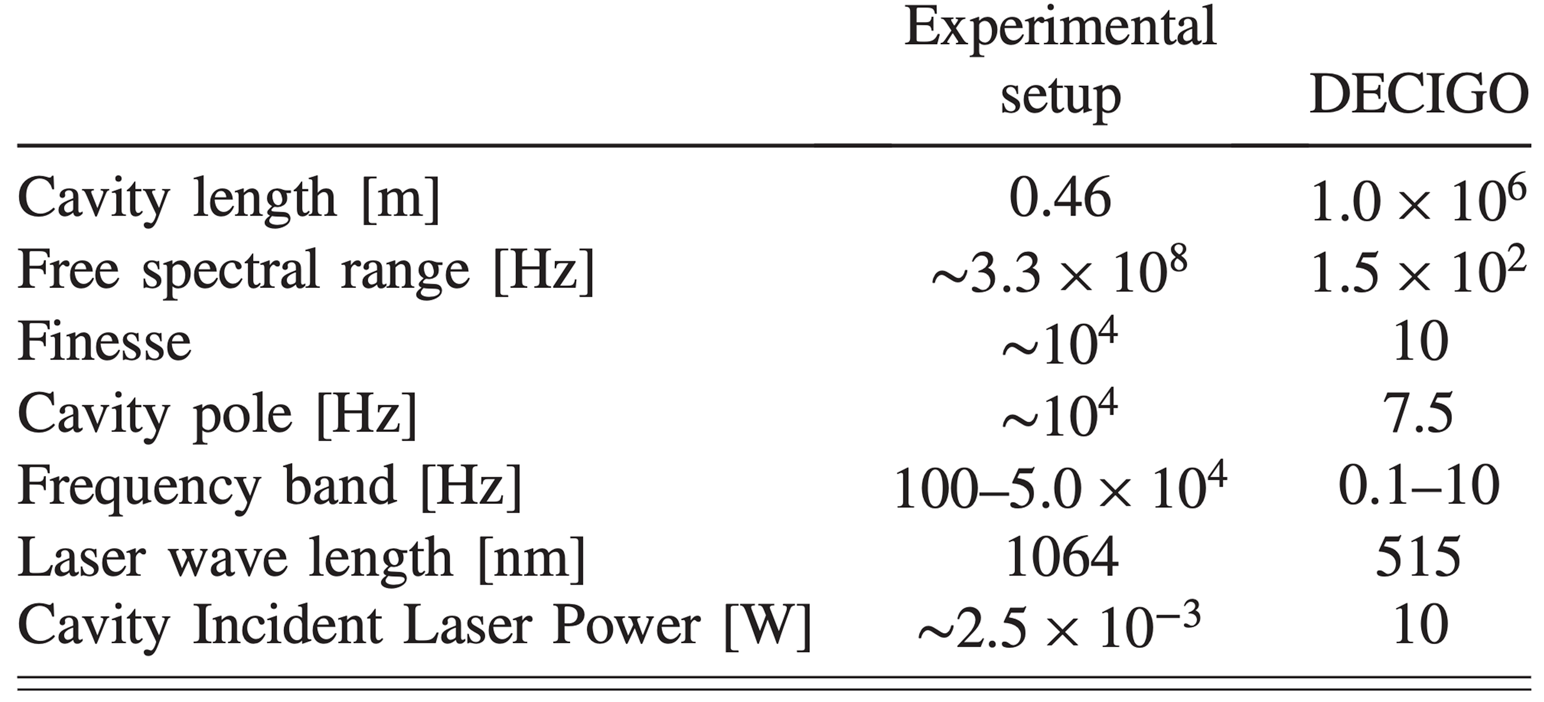
The primary advantage of the BLFPI method is that it does not require the ultra-precise cavity length control required in conventional methods*5. Additionally, since it is not necessary to maintain a constant cavity length, the system can tolerate free expansion and contraction of the cavity length during operation. This relaxes the requirements for controlling the satellite separation distance, potentially reducing propellant consumption and contributing to long-duration observations.
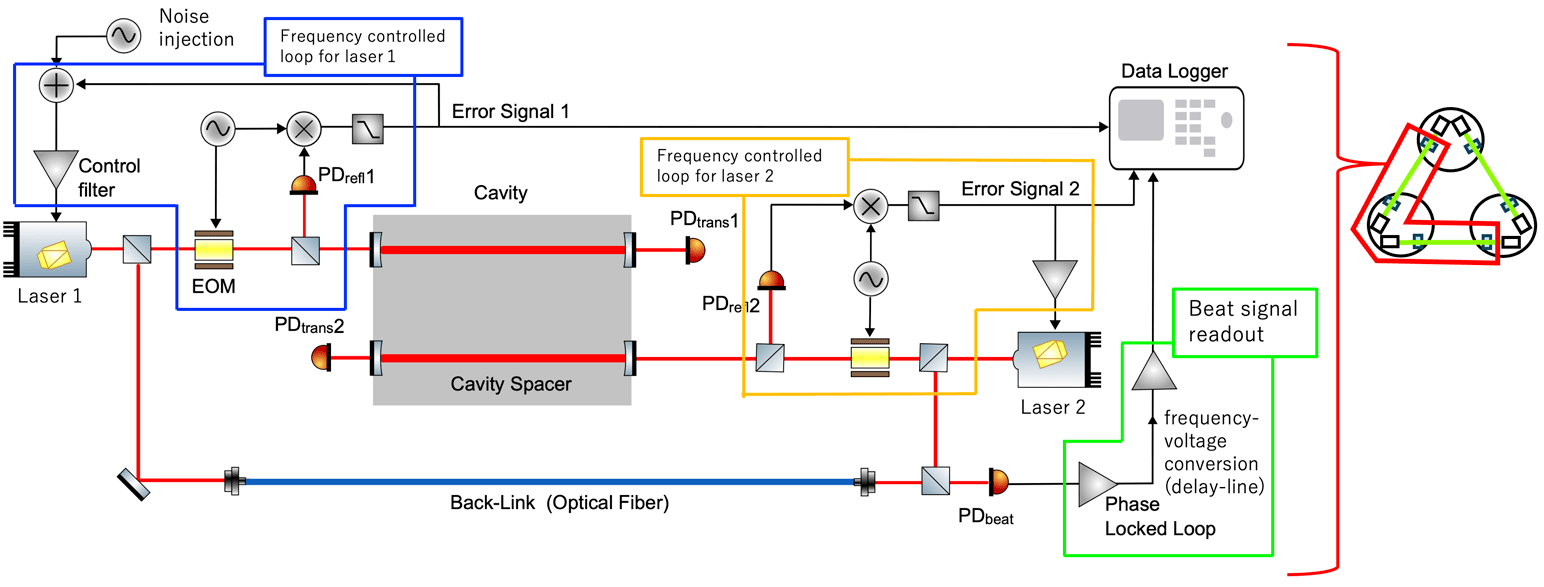
One challenge of the BLFPI is the contamination of the beat signal by the laser frequency noise. The laser frequency is initially stabilized before incident to the cavity and then further stabilized to follow the cavity. However, for missions like DECIGO, an additional frequency noise suppression of approximately four orders of magnitude is required*6. To address this issue, a method called the "subtraction method" has been theoretically proposed, wherein signals obtained from each part of the interferometer are combined post-processing with the cavity response to subtract the frequency noise. However, this method had not been experimentally demonstrated.
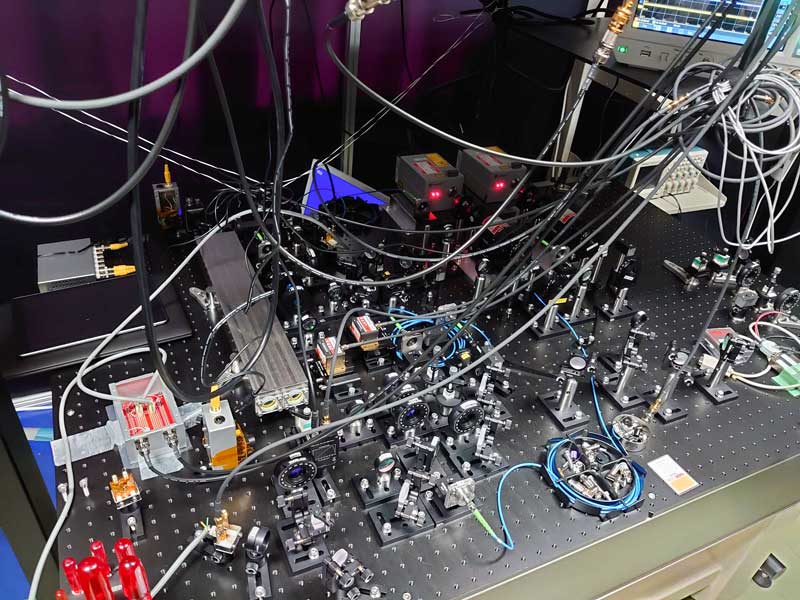
In this research, we constructed a tabletop BLFPI experimental setup simulating the spacecraft and demonstrated the reduction of the laser frequency noise on real data obtained from the setup. A notable aspect of this demonstration is that noise subtraction was achieved even in the frequency band where the low-pass frequency response, characteristic of the cavity, known as the cavity pole*7, was observed. Since the cavity pole frequency falls within the observation band of actual detectors like DECIGO, demonstrating noise subtraction around this frequency band is of significant importance. Table 1 shows the correspondence between this setup and DECIGO.
Figure 2 presents a schematic of the experimental setup, and Figure 3 shows a photograph of the actual setup. The experimental setup corresponds to a single spacecraft and the mirror segment of its counterpart spacecraft, as shown in the red frame on the right side of Figure 2. It consists of two cavities, two lasers and a control system that resonates them in a cavity, and the back-link interferometer to obtain the beat signal between the lasers. If the cavities are scaled down to laboratory size while maintaining the same design as the spacecraft, such as the reflectivity of the mirrors, the cavity pole frequency which corresponds to the travel time of light in the cavity, becomes very high, making realistic measurement difficult. To compensate for the reduced scale compared to the spacecraft and ensure that the cavity pole frequency falls within a practical demonstration band, the cavities employ highly reflective, low-loss mirrors to achieve sharp resonance and increase the number of light round trips. Furthermore, both cavities are integrated into a single low-expansion metal spacer, allowing for the common-mode rejection of environmental disturbances such as acoustic and temperature variations, enabling the verification of minute noise levels when the beat frequency is extracted.
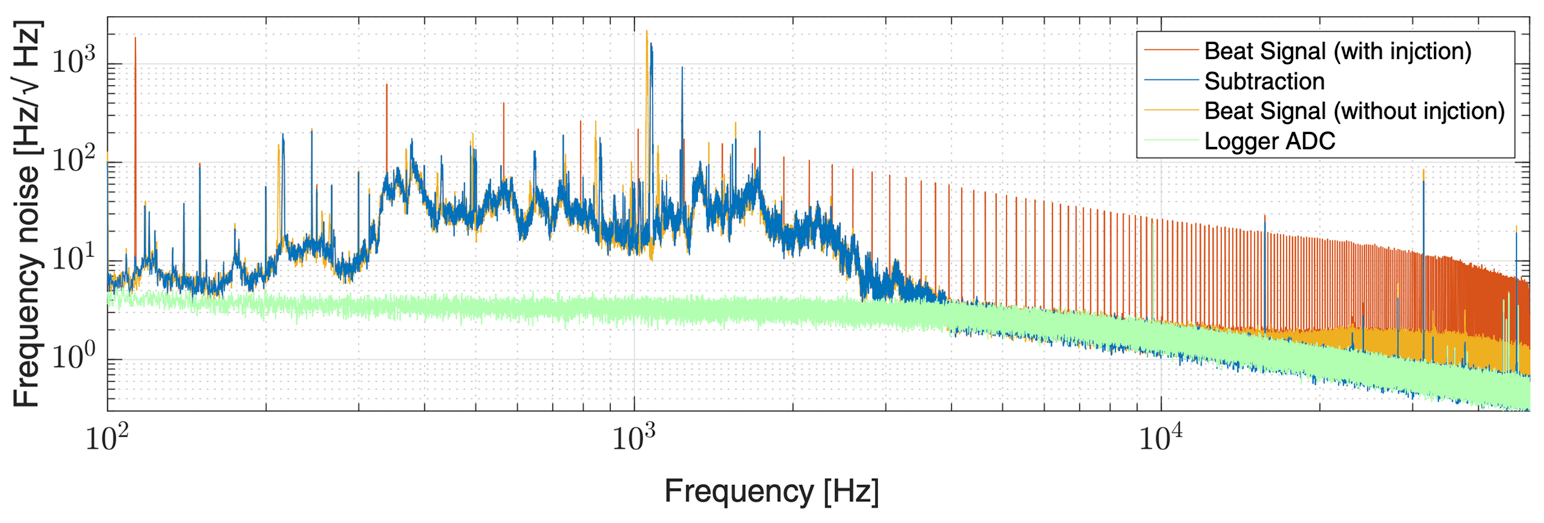
Figure 4 presents the frequency noise spectrum of the beat signal measured in this experiment. To effectively demonstrate, the laser frequency was artificially excited to generate significant noise, which was then shown to be reducible. A square wave with a frequency of 113 Hz was used as the excitation signal, leading to the appearance of peaks at odd harmonics of 113 Hz in the plot before subtraction. In contrast, the plot after subtraction shows that the injected peaks were successfully removed, including those in the frequency band beyond the cavity pole. The maximum noise reduction ratio achieved at the fundamental frequency of 113 Hz was 188±29. Additionally, in the original beat spectrum, the laser's inherent frequency noise was nearly invisible due to environmental noise in the laboratory, but in the high-frequency region, the effect of the original laser frequency noise emerged. Since the after-subtraction spectrum in the high-frequency region is reduced below the level before signal injection, it was confirmed that not only the injected signal but also the original laser frequency noise was subtracted.
Furthermore, the original thesis provides a quantitative evaluation of the residual noise after subtraction and estimates the contribution of various factors that may explain the experimental residuals, clarifying points for improvement toward achieving the target noise reduction ratio of 104. This demonstration represents a significant milestone in the practical implementation of the BLFPI method and contributes to the development of space-based optical cavity detectors by introducing new technological diversity and improving feasibility. The results are meaningful in that they may lead to Japan's leadership in high-sensitivity gravitational wave observation in the 0.1 Hz band in the future.
Terminologies
- *1 Expected Scientific Outcomes from Observing Gravitational Waves in the 0.1 Hz Band: Over 90 gravitational wave event candidates have been reported by ground-based laser interferometer detectors, such as Advanced LIGO, in the United States. These events correspond to frequencies around 10-1000 Hz and originate from binary systems of stellar-mass black holes or neutron stars. On the other hand, observing in the lower frequency band of approximately 0.1-10 Hz could enable new scientific achievements. For example, it would allow the early detection and localization of neutron star binaries months before their merger. This early detection could facilitate multi-messenger observations by sharing information with electromagnetic telescopes. Additionally, the potential to detect heavier intermediate-mass black holes, ultimately, the direct observation of primordial gravitational waves generated the early universe.
- *2 Space-based detectors: It is known that ground seismic noise increases in the low-frequency band. On the other hand, since the vibration isolation using pendulums, which is mainly used in ground detectors, does not work effectively in the low-frequency band, it is difficult to achieve high sensitivity. In addition, in the future, fluctuations in the gravitational field (gravitational gradient noise) derived from ocean and atmospheric fluctuations may also become an issue. In order to essentially avoid these terrestrial-origin noises, there are plans to construct detectors in space. There are projects such as LISA in Europe and the United States, which aims to detect in the 0.1 mHz-100 mHz band, and DECIGO in Japan, which targets the 0.1-10 Hz band.
- *3 Fabry–Pérot cavity: The mechanism that makes the resonance of the laser between the mirrors is called a cavity. A cavity with mirrors arranged in a straight line is called a Fabry–Pérot cavity. The state in which the distance between the mirrors is exactly a positive multiple of half the wavelength of the laser being input is called resonance. In a resonant state, many waves overlap, and light bounces back and forth many times. If the mirror position or laser frequency changes near the resonant state, the resonant conditions are disrupted, and a steep change occurs in the reflected or transmitted light from the cavity, so it can be used as a highly sensitive sensor.
- *4 BLFPI-type space detector: Proposed by JAXA and NAOJ researchers in 2021. You can watch an animation explaining the concept via the link below.https://jpsht.jps.jp/article/1-054/
- *5 Conventional Fabry–Pérot type space detector: The method involves equipping each spacecraft with a single laser light source and separating it into two directions, and there are methods such as Differential Fabry–Pérot and Fabry–Pérot Michelson. Because a common laser source is used, there are advantages such as the common-mode rejection of frequency noise, which is a problem with BLFPI, when acquiring differential signals. On the other hand, because there are two cavities for the laser in this system, it is not possible to control both cavities using the laser frequency alone, and it is also necessary to control the cavity length. At this time, it is necessary to control the cavity length of around 100 km to 1000 km with nanometer-level accuracy, which is also a very challenging. This method is being developed in parallel with the BLFPI.
- *6 Requirements for frequency noise: For example, the target sensitivity of B-DECIGO, which is a DECIGO outpost satellite, is 2×10-23 /√Hz at 1 Hz. Since B-DECIGO is planned to use an iodine-stabilized laser with a wavelength of 515 nm, it is necessary to achieve a frequency noise of around 10-9 Hz/√Hz (The signal-to-noise ratio is assumed 10 for the target sensitivity). The initial stability of the stabilized laser is expected to be around 0.1 Hz/√Hz, and this will be further stabilized by feedback control to the main cavity. At this point, the suppression gain is thought to be around 104-105, so the frequency noise at this point will be around 10-6-10-5 Hz/√Hz, and a further 3-4 orders of noise reduction will be required to achieve the target.
- *7 Cavity pole frequency: In an optical cavity, light bounces back and forth inside the cavity many times, leading to a low-pass-like frequency response. The frequency corresponding to this cutoff is called the cavity pole frequency. In this demonstration, we estimated the cavity response and implemented it in the subtraction process, and confirmed that it was possible to reduce not only in the flat low-frequency part below the cavity pole frequency, but also in the frequency band around the cavity pole frequency and above it.
Information
Journal Title | PHYSICAL REVIEW D |
---|---|
Full title of the paper | Experimental demonstration of back-linked Fabry–Pérot interferometer for a space gravitational wave antenna |
DOI | https://doi.org/10.1103/PhysRevD.109.022003 |
Publish date | 10 January 2024 |
Author(s) | Ryosuke Sugimoto, Yusuke Okuma, Koji Nagano, Kentaro Komori, and Kiwamu Izumi |
ISAS or JAXA member(s) among author(s) | SUGIMOTO Ryosuke (Department of Space and Astronautical Science, School of Physical Sciences, Graduate Institute for Advanced Studies, SOKENDAI, and Department of Space Astronomy and Astrophysics, ISAS), OKUMA Yusuke (Department of Space Astronomy and Astrophysics, ISAS), NAGANO Koji (Department of Space Flight Systems, ISAS), KOMORI Kentaro (Department of Space Astronomy and Astrophysics, ISAS), and IZUMI Kiwamu (Department of Space Astronomy and Astrophysics, ISAS). |